Specifying concrete repair
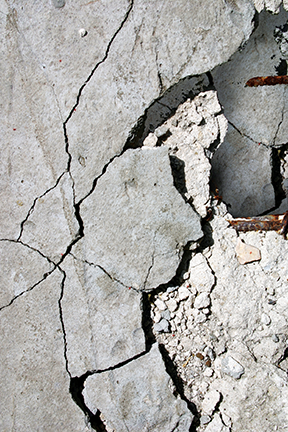
By Alexander M. Vaysburd and Benoît Bissonnette
Good concrete repair is not a bandage ‘fix’ for a structure in trouble—rather, it is a complex system that consists of numerous engineering tasks (Figure 1). Designing and specifying concrete repair has unique needs differing from new construction. Thus, the specifications must serve as action plans or roadmaps for the project’s engineer, contractor, and quality controller.
Unfortunately, when it comes to the durability of repaired concrete structures, project documentation is not always adequate. For example, one shortcoming constantly repeated in specifications is the frequent reference to the “direction” of the engineer or architect. The authors came across a specification concerning a material for an industrial plant repair project that simply read:
The patch repair material’s durability shall be as directed by the engineer.
The subjective character of such specifications can make appropriate bidding impossible. Additionally, such references are useless because they stem from either not knowing what should be required, or from a refusal to make an effort to study and analyze the issue to determine a specification. It is an unfortunate fact some “directing” engineers can be literal to a degree the specifier never intended.
Many specifications for repairs are a mixture of referenced standards and ‘cut-and-paste’ clauses from previous projects, recycled with little thought. Achieving durability should be found in carefully considered specifications for a particular project. However, this does not appear to be the case—widespread durability problems have led to extensive repair of previous repairs and, in some cases, eventual replacement of structural members.
Durability considerations
There are several durability-related factors playing into concrete repair specifications.
Compressive strength
There is a common misconception higher-strength cement-based materials are necessary in severe environments for enhanced durability. However, this way of thinking does not take into account the realization a stronger and stiffer material is more likely to crack because the higher modulus of elasticity increases the tensile stress arising from shrinkage and other restrained volume changes.
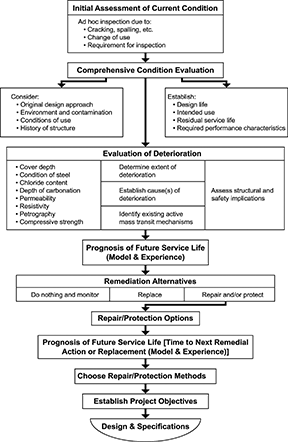
Unfortunately, many specifiers blindly opt for so-called high-strength (i.e. ‘high-performance’) cementitious materials containing ingredients such as silica fume or high-range water-reducing admixtures (HWRAs), which can also have negative side effects on performance of repair materials. While useful in certain applications, some components can also have negative side effects when it comes to using them for repair work. The terms ‘high-performance’ and ‘high-strength’ are often used as synonyms. However, high compressive strength is not an indication of improved durability and performance—in fact, the increase in strength may be gained at durability’s expense.
In reviewing 120 North American projects, the authors could not find a specification for a repair material with a compressive strength of approximately 20 MPa (3000 psi), even when the strength of the existing substrate was of such magnitude. The rationale seems to be the belief a 55-MPa (8000-psi) ‘high-performance’ material will always be more crack-resistant and achieve better durability.
The major fault of any material is not a matter of strength or stiffness, but rather a lack of resistance to crack initiation and propagation. The Victorians had high-strength cast iron, but its brittleness led to many failures. They soon realized ductility was the essence of safe structures and began using lower strength, ductile mild steel. In many cases, it makes sense to follow their lead and pay more attention to deformability and crack resistance of cementitious repair materials.
Another critical problem in many specifications is the often unjustifiable requirement for high early-strength repair materials. This may be necessary for some special applications, but for typical repairs it creates a greater potential for higher shrinkage and cracking. Long-term durability is primarily achieved by dimensional stability, not by high early-strength. Accelerated gain in strength generally comes with more self-stress from drying shrinkage, autogenous shrinkage, and thermal contraction. The rate of strength gain, in addition to the total degree of hydration, has a significant effect on cementitious materials’ pore structure, micro- and macro-cracking, and transport (i.e. permeability) properties.
Accelerated strength gain has also been known to result in lower ultimate strength. However, the effect on durability is generally overlooked. At a ‘normal’ rate of strength gain (i.e. three days for 50 per cent ultimate strength, seven days for 70 per cent, or 28 days for 100 per cent), hydration products have sufficient time to diffuse throughout the cement matrix and precipitate uniformly. At accelerated rates, hydration is so much faster than the diffusion process that most products remain static near the cement grains, leaving the interstitial space relatively open.